Nanoferroics State of Art Gradient Driven Couplings and Advanced Applications
Abstruse
Since its inception more than 25 years agone, Piezoresponse Forcefulness Microscopy (PFM) has become one of the mainstream techniques in the field of nanoferroic materials. This review describes the development of PFM from an imaging technique to a ready of avant-garde methods, which have played a critical role in launching new areas of ferroic research, such as multiferroic devices and domain wall nanoelectronics. The newspaper reviews the impact of advanced PFM modes apropos the discovery and scientific agreement of novel nanoferroic phenomena and discusses challenges associated with the right interpretation of PFM data. In decision, it offers an outlook for futurity trends and developments in PFM.
Introduction
The invention of the atomic force microscope (AFM) in 1986 marked a dramatic shift in scientific research by providing a multifunctional toolbox to explore and manipulate functional properties of a wide range of materials at the nanometer scale. For ferroelectrics and other polar materials, the introduction of i of the voltage-modulated versions of AFM—piezoresponse forcefulness microscopy (PFM)—has produced a wealth of new opportunities. PFM enables non-destructive visualization and command of FE nanodomains, as well equally direct measurements of the local physical characteristics of ferroelectrics, such as nucleation bias, piezoelectric coefficients, disorder potential, energy dissipation, and domain wall (DW) dynamics (see Box i). With this, PFM has essentially driven the whole field into the realm of the nanoscale1.
Since the publication of the kickoff review book2, the experimental and concrete principles of PFM operation have become common knowledge. A number of papers and books provided a comprehensive description of its technical details and gave abundant examples of PFM imaging and modification capabilities3,4,5. Equally the field matured, new advanced PFM modes were adult (Box 2). However, the broad awarding of PFM revealed a growing number of challenges and concerns related to the imaging mechanism, information interpretation, and quantification. While nanoscale domain imaging has been crucial for the initial advent of nanoferroelectric research, it became likewise clear that a more conscientious assay of the PFM image formation machinery was necessary, forth with comprehensive information on the structure, physics, and chemistry of the materials nether investigation, to distinguish real effects from artifacts.
This article, instead of describing the experimental issues of PFM and a multifariousness of accumulated data, focuses on new science and discoveries enabled by PFM. After presenting a cursory historical overview of the development of conventional PFM into a set of advanced modes, it describes the function of PFM in exploration of new emergent phenomena, including DW conductivity, magnetoelectric switching, voltage-gratis flexoelectric domain control, tunneling electroresistance, domain vertices, and polar vortices. Specific attention is paid to challenges in PFM application related to a variety of electromechanical coupling phenomena and the complex image formation mechanisms. Based on the recent advances and challenges in the field of nanoferroics and other functional materials, this review offers an outlook for future developments and trends in PFM.
A brief history of PFM
A key element of AFM that brought about the possibility of high-resolution label of materials is the highly localized interaction between the probing tip and the sampleone,ii. In the case of ferroelectrics, a broad range of polarization-dependent backdrop, such as elastic, structural, electrochemical, pyroelectric, and piezoelectric, makes them a perfect cloth system for testing various AFM-based methods sensitive to the electrostatic, mechanical, and/or electromechanical (EM) tip–sample interactions4. Application of PFM to ferroelectrics makes utilize of their piezoelectric action and its linear coupling with polarization in the depression-field range. The underlying physical basis of PFM is similar to the idea of piezoelectric polarization-sensing proposed by Kaufman in the belatedly 1950s6. Owing to the converse piezoelectric consequence, application of an a.c. electric field to a ferroelectric sample results in surface vibration. The polarization state can be determined by measuring the magnitude and phase of this piezoelectric vibration signal. Therefore, the term "piezoresponse" was initially coined for this AFM-based technique7 although, as it was shown afterward, the EM coupling machinery detected by PFM tin can go well across the piezoelectric effect8. The first AFM imaging experiments based on the antipodal piezoelectric effect were performed by Guethner and Dransfeld in 1992; their paper on local poling of the ferroelectric polymer vinylidene-fluoride trifluoroethylene (PVDF-TrFE) tin can be considered every bit the nascence of PFMnine. However, even earlier this seminal newspaper, Dransfeld's grouping had used scanning near-field acoustic microscopy to notice dissimilar piezoactivity of the poled and not-poled regions of PVDF-TrFE filmsten. The lateral resolution of ane µm, quite impressive for that time, was determined by the size of the probe apex. A twelvemonth before, the same group used scanning tunneling microscope for nanoscale piezoelectric measurementseleven. However, this approach was too challenging to implement due to the loftier instability of the feedback signal. Thus, attempts to use the piezoelectric consequence for local polarization detection were well under way from the early 1990s, building up on concrete principles and technical approaches proposed earlier. Yet, it took several years for PFM to take off and start its way toward condign a mainstream imaging and analytical tool for ferroelectrics. The PFM modes integrated into commercial AFM systems started to appear on the marketplace in the tardily 1990s (Seiko Instruments, Park Scientific Instruments).
Historically, the invention of PFM is closely related to the advances in ferroelectricity, which, since its discovery in the 1920s, has passed through a number of well-marked "phases" when specific materials, techniques, or models were peculiarly popular12. Even earlier the development of scanning probe microscopy, it was predicted that the 1990s would be the age of "miniaturization," when the scaling beliefs of FEs would be at the forefront of scientific studies. PFM, along with advances in fabrication of functional ferroelectric nanostructures and theoretical modeling, turned this prediction into reality. Nanoscale visualization of the every bit-grown domain construction in Lead(Zr,Ti)Oiii (PZT) thin filmsthirteen and the demonstration of polarization control in individual PZT nanocellsxiv marked the offset of a widespread employ of PFM and launched the era of nanoferroelectric research. Application of PFM to ferroelectric structures with sub-µm dimensions, such equally ultrathin epitaxial films15, nanoscale capacitors16, and nanotubes17, opened a possibility for direct studies of the intrinsic and extrinsic mechanisms of their scaling beliefs. The fast progress in PFM applications was further additional by the demonstration of local spectroscopy measurements—PFM hysteresis loops13,18, which allowed nanoscale testing of physical parameters, such every bit nucleation bias, imprint, piezo-coefficients, and disorder potential. This voltage-dependent PFM approach afterward evolved into switching spectroscopy PFM (Box 2)—an effective tool for two-dimensional (2D) nanoscale mapping of the local switching characteristics19. An important step forwards in nanoferroelectric research was the visualization of domain structures through the top electrode20. This evolution allowed the direct observation of domain nucleation and DW dynamics at the sub-100-ns time calibration in device-grade PZT capacitors using stroboscopic PFM (Box 2) and provided invaluable information on the scaling beliefs of ferroelectric random admission memory devices (FeRAM)21.
Simultaneous detection of electrically induced contraction/expansion and shear deformation of the sample—the approach later termed every bit vector PFM (Box ii)22—allowed delineation of the in-airplane and out-of-plane polarization components and three-dimensional (3D) polarization reconstruction23. Expanding usage of PFM stimulated serious theoretical efforts to describe the image germination mechanism as well as tip-induced domain switching, and to sympathise the meaning of local PFM hysteresis loops 24. Detailed analysis of the sample response and cantilever motility revealed limitations of PFM as a quantitative technique, acquired by the nonuniform distribution of the tip-generated electroelastic fields, lack of information on the tip geometry, and complex tip–sample contact mechanics, in improver to the non-trivial sample symmetry/orientation-dependence of the PFM signal25.
The mid-00s marked a growing utilize of PFM in combination with other microscopy techniques further expanding the frontiers of the nanoferroic field. High-resolution imaging of both antiferromagnetic and ferroelectric domains in multiferroic BiFeO3 by X-ray photoemission electron microscopy (PEEM) and PFM, respectively, led to the first demonstration of electrical control of antiferromagnetic domains in a single-phase multiferroic26. In i of the kickoff examples of simultaneous use of PFM and conducting AFM (C-AFM), Yoshida et al. demonstrated polarization-modulated conduction in PZT thin films27. This arroyo has been utilized to its full extent during the experimental observations of the electroresistance result in ferroelectric tunnel junctions28,29 and enhanced conductivity of ferroelectric DWsthirty. A breakthrough discovery of alternating net magnetic moments at ferroelectric DWs in multiferroic hexagonal manganites has been accomplished by using PFM in conjunction with magnetic force microscopy (MFM)31.
Around the same time, the utility of PFM in testing the nanoscale EM beliefs of a broader range of materials, such as piezoelectric semiconductors and biomaterials, was established. Intrinsic piezoelectricity of biopolymers enabled outset nanoscale structural imaging of calcified and connective tissues as well as orientational imaging of poly peptide molecules32. Similarly, the polar structure of III–5 semiconductors allowed delineation of the inversion domains associated with unlike termination layers32. Extension of PFM usage to materials with weak EM properties necessitated enhancement of PFM sensitivity, which was addressed by the development of the resonant-enhanced mode of PFM by Rodriguez et al. in 2007 (Box ii)33 preceded past work of Harnagea et al.34.
PFM is still evolving: for case, loftier-speed and multi-dimensional data conquering components have been recently added to its already wide portfolio of capabilities. Latest advances are related to the development of metrological PFM and band-excitation PFM (Box two), which allow multi-frequency spectroscopic analysis of the dynamic processes in ferroelectrics.
Challenges of PFM
The conceptual simplicity of PFM and the ease, with which the PFM mode tin be operated on modern AFMs, should non be mistaken for triviality of PFM data analysis and interpretation. AFM systems tend to plow more and more into "black boxes" that readily produce information with large throughput and high accurateness. On the 1 mitt, the "black-box" pattern can be an outcome for specialists because of reduced flexibility and rather limited options for the development of new experiments. On the other hand—and more problematically—new users are no longer required to accept a thorough knowledge or understanding of the experimental details to record PFM data. As a consequence, the danger of misinterpretation and scientifically incorrect conclusions increases. In particular, it is important to remember that PFM is non a foolproof verification tool for ferroelectricity: PFM detects the electrically modulated mechanical strain—a property that is necessary but not sufficient for determining the ferroelectric behavior. The reason is that, in addition to the polarization-dependent piezoelectric deformation, in that location are several other mechanisms that can lead to a ferroelectric-like PFM response. These mechanisms are discussed below along with other challenges associated with successful experimental implementation and right estimation of PFM data.
Image formation
Independent of a specific PFM mode, image germination always follows the same basic principle. The response of the sample is probed by the tip and recorded with the help of lock-in technique, translating the tip-oscillation into a voltage. The output voltage is then used to generate 2D maps by scanning the tip line-by-line across the sample. PFM is thus by no means a direct measure of ferroelectricity or piezoelectric deformation. Agreement whether the detected signals stand for to the intrinsic ferroelectric properties (Fig. 1a) or potential artifacts is a major challenge.
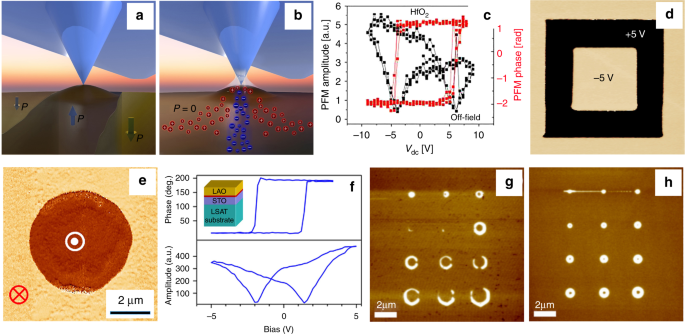
Ferroelectric and non-ferroelectric contributions in PFM. a Attributable to the linear coupling betwixt piezoelectric activity and spontaneous polarization in the depression-field range, ferroelectric domain imaging tin be carried out by detecting the electrically induced surface deportation due to converse piezoelectric effect. b In add-on to the mechanism mentioned in a, other mechanisms may contribute to the prototype formation in PFM in ambient conditions: electrochemical reactions facilitated by the water meniscus at the tip–sample junction, field-induced migration of anions/cations, surface charging, and carrier injection, as well as non-linear high-order strain effects. c Switching spectroscopy PFM on amorphous not-ferroelectric HfOtwo films yields hysteretic behavior in the PFM phase and amplitude signals. (Reprinted with permission from ref. 42. Copyright 2015 American Chemic Society). As both ferroelectric and not-ferroelectric materials can showroom hysteretic beliefs in switching spectroscopy PFM, the observation of such loops lonely is insufficient to confirm the ferroelectric behavior. d Non-ferroelectric signals due to accuse injection and electrostatic forces tin mimic the ferroelectric behavior in PFM measurements of non-ferroelectric LaAlO3 films. This paradigm demonstrates reversible and long-living PFM phase contrast, which arises after applying a ±5 V d.c. bias to the tip. The image size is viii × 8 µm2. (Reprinted from ref. 35, with the permission of AIP Publishing). e Electrically switchable and stable PFM phase contrast in Pt/LaAlO3/SrRuOiii capacitors is attributed to electrically induced oxygen vacancy migration. (Reprinted with permission from ref. 81). f Redistribution and aggregating of oxygen vacancies either on the surface or at the interface tin can crusade hysteretic field-dependent beliefs of the PFM bespeak in LaAlO3/SrTiOiii heterostructures. (Reprinted with permission from ref. 36. Copyright 2012 American Chemical Gild). g, h Effect of environment in PFM manifests itself in creating different boundary conditions for domain writing and retention. PFM images of domains generated by voltage pulses under ambience conditions (g) and depression humidity (h) reveal drastically unlike domain sizes and shapes (chiliad, h are both reprinted from ref. 47, with the permission of AIP Publishing)
Origin of the electromechanical response
Understanding the mechanism behind the EM signal is a key issue for correct data estimation. For example, accuse injection and field-induced oxygen vacancy migration can result in a switchable internal electric field that biases the intrinsic electrostriction, producing a switchable PFM response35,36. Electrochemical strain due to ionic migration (Fig. 1b)37 is likewise relevant for mixed ionic–electronic conductors, biosystems, and polymers38. In principle, any material that exhibits a macroscopic D–Due east hysteresis loop tin can display hysteretic behavior in PFM39. College-order EM effects, such as flexoelectricity and switchable electrostriction, can also influence PFM image germination.
Verification of ferroelectricity
To evidence ferroelectricity, two effects have to be verified: the beingness of domains with unlike orientation of polarization and hysteretic switching betwixt the contrary domain states by electric fields. In PFM studies, information technology has become standard to nowadays images taken before and after electrical poling to show the beingness of switchable polarization domains, while PFM switching spectroscopy is commonly used to measure out the corresponding hysteresis loop. However, in PFM, the non-piezoelectric effects mentioned above can assuredly mimic the ferroelectric beliefs, producing long-lasting bistable states resembling written domains equally well equally hysteresis loops even in non-ferroelectric materials38. An increasing number of studies on non-ferroelectric materials, for instance, TiOtwo 8 and LaAlO3/SrTiO3 36, clearly demonstrate that PFM measurements are insufficient to ostend the ferroelectric beliefs (Fig. 1c–f). A hit illustration of PFM limitations in this affair is the ongoing controversy related to the machinery of the polar behavior in organic perovskites40,41. A scanning probe microscopy-based approach has been recently proposed as a possible mode to identify PFM artifacts and distinguish between the ferroelectric and non-ferroelectric PFM contributions42.
Calibration
While thorough signal calibration and careful separation of vertical (buckling/deflection) and horizontal (torsion) cantilever excitations allows 3D mapping of polarization, PFM cannot be considered equally a quantitative tool for measuring the local piezoelectric coefficients. This limitation is due to a variety of reasons, i.e. non-uniform field distribution, contributions from the not-local electrostatic furnishings and multiple piezoelectric tensor elements to the EM displacement at a given point; mechanical constraints from the immediate (not-activated) surrounding; unknown contact resistance; etc43. In addition, system-inherent groundwork contributions can have a significant impact on the PFM signal44. Quantitative PFM evaluation of the piezoconstants can be performed in a limited number of cases, for example, for d33 measurements in perovskite (001)-oriented capacitors with a small thickness/area aspect ratio22.
Environmental influence
In improver to the aforementioned intrinsic phenomena, the sample environs and surface effects can be decisive for the effect of a PFM browse45. Nether ambient weather condition, a water meniscus at the tip–sample contact promotes surface charging and electrochemical reactions, affecting the domain switching and retentiveness behavior (Fig. 1g, h)46,47.
Frictional forces
Electrically driven shear deformations, which represent to the off-diagonal elements of the piezoelectric tensor, can exist detected past monitoring the lateral PFM indicate induced via tip–surface friction forces. However, surface contamination, topographical features, inhomogeneous electrical fields, complex frequency spectra, and mechanical clamping can significantly complicate the estimation of lateral PFM data and cause imaging artefacts (Fig. 2). Equally these phenomena collectively affect the friction forces, the lateral PFM indicate is a superposition of various contributions rather than a straight fingerprint of the Iron domains. This makes lateral PFM essentially unsuitable for studying domains in the ferroelectric capacitors (Fig. 2a, b). Particular caution is required when using lateral PFM to analyze ane-dimensional and 2d structures with constrained geometry, including nanoparticles, nanorods, and nanotubes (Fig. 2c, d).
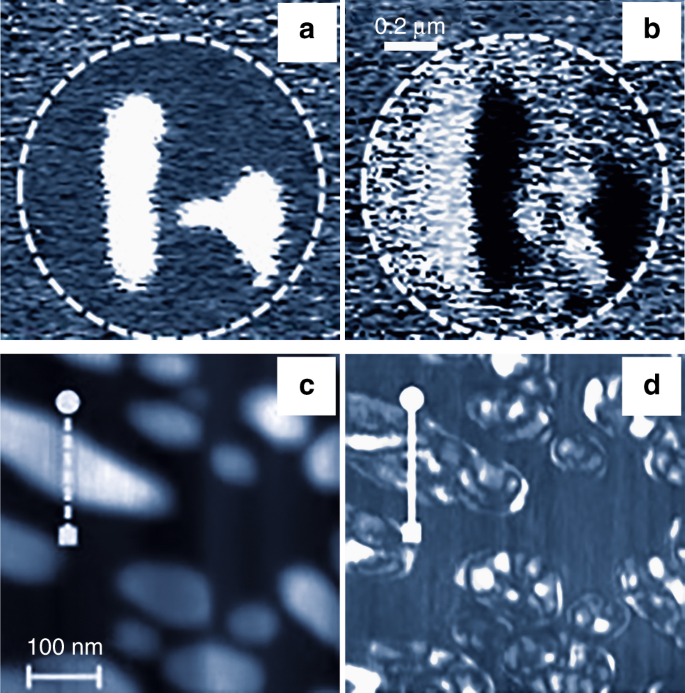
PFM imaging artefacts. a, b Vertical (a) and lateral (b) PFM stage images of the 180° domains in a microscale (001)-oriented Pb(Zr,Ti)O3 capacitor. Lateral PFM contrast in b arises due to the changes of the top electrode morphology induced by the vertical expansion and contraction of the antiparallel 180° domains causing torsional motility of the cantilever, which changes its direction with the alter in the surface gradient sign. c, d Any asymmetry in the tip–sample system, for case, due to the surface gradient, asymmetric tip apex, local variations in sample stoichiometry, or dielectric or rubberband constants, may cause imaging artifacts specially pronounced in lateral PFM. c Diminutive force microscopic topography and d lateral PFM images of the (001)-oriented Lead(Zr,Ti)O3 nanograins showing strong variations in the lateral electron microscopic response, which are not related to the in-plane polarization (c, d are adjusted from ref. 99, with the permission of AIP Publishing)
Standards on PFM measurements
So far, attempts to develop a standardized PFM measuring protocol to proceeds consequent imaging and spectroscopic results were unsuccessful due to the complication of the tip–sample interaction and variability of the imaging germination mechanisms as explained above. Although PFM measurements are carried out in a similar style irrespective of the tested materials, PFM practitioners apply a wide range of imaging parameters fifty-fifty for the aforementioned type of samples: for example, the reported imaging frequencies are in the range from tens to hundreds of kHz. Furthermore, although the conductive cantilevers typically used for Atomic number 26 testing take rubberband constants of several Due north/m, the modulating bias can vary from hundreds of mV up to several Volts. Coordinated efforts by the PFM users through the circular-robin comparative spectroscopic testing of the standardized samples, such every bit periodically poled stoichiometric LiNbO3 crystals or (001)-oriented single-crystalline PbTiO3 or PZT thin picture show capacitors, using PFM in conjunction with laser Doppler vibrometry seem to be necessary to identify the data variations owing to different experimental conditions and setups.
Discovery of new phenomena and testing polarization-enabled functionality
While at the earlier stages of its use PFM was essential for achieving a nanoscale insight into the ferroelectric switching phenomena, more recently, its combination with complementary nanoscale characterization tools allowed exploration of emerging phenomena in nanoferroic systems. This led to several key discoveries revealing unique electronic, magnetic, and transport properties of nanoferroics (Fig. three).
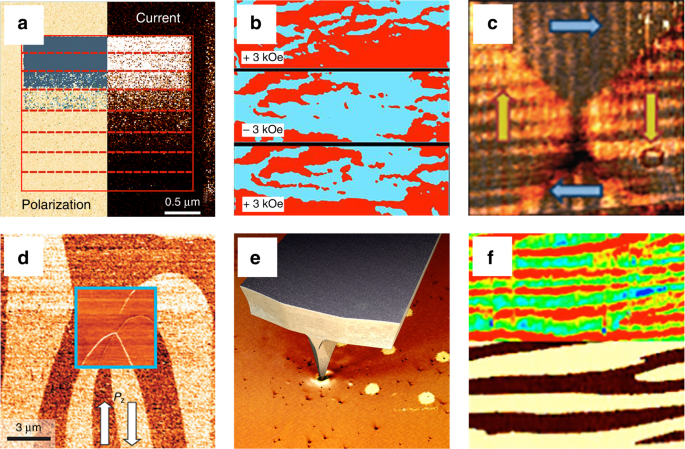
PFM and discovery of novel phenomena. a Tunneling electroresistance effect verified by spatially resolved correlation between the onset of polarization reversal revealed by PFM and a resistive switching imaged by C-AFM. A alter in PFM contrast correlates with the transition from low current (nighttime contrast) to high current (vivid contrast). (Adapted with permission from ref. 29. Copyright 2009 American Chemical Society). b Magnetic field control of ferroelectric domains in the multiferroic solid solution of atomic number 82 zirconium titanate and lead iron tantalite (PZTFT): PFM images reveal a change in the ferroelectric domain structure, which depends on the orientation of the external magnetic field. (Adapted by permission from Springer Nature: ref. 62). c Emerging topologic domain structures: flux-closure domain formation in a microscale single-crystalline BaTiO3 lamella revealed by vector PFM. The paradigm size is 2 × 2 µm2. (Reprinted past permission from Springer Nature: ref. 66). d Domain wall conductivity: the 6 × 6 µmtwo lateral PFM epitome obtained on a ErMnOiii crystal with arrows indicating the in-plane polarization direction. The inset shows a C-AFM image acquired at the same location revealing different conductance for dissimilar types of domain walls. (Reprinted by permission from ref. 69). due east Flexoelectric control of polarization: in ultrathin ferroelectric films, a strain gradient, generated by PFM tip pressure, tin can produce a flexoelectric field high enough to exceed the coercive field of the film resulting in purely mechanical switching of nanoscale domains. f Enhancement of photovoltaic current (C-AFM prototype in the upper panel) at domain walls (PFM phase paradigm in the lower panel). The size of each panel is 3.0 × 1.5 µmtwo. (Adapted from ref. 86 under the terms of the CC-BY 4.0 license)
Tunneling electroresistance
One of the most exciting discoveries, involving the employ of dual a.c. resonance tracking PFM, was the electroresistance effect in ferroelectric tunnel junctions (FTJs)29,48 followed past the sit-in of Atomic number 26 command of the carrier spin polarization in nanoscale capacitors49. PFM demonstrated that polar displacements could be stable and switchable in ultrathin films with a thickness down to but several unit cells and established a direct correlation between ferroelectric switching and the electroresistance upshot (Fig. 3a). PFM detection of fractional polarization switching associated with unlike tunneling-current levels led to the discovery of the memristive beliefs in FTJs50,51 opening a possibility for the development of multilevel memories and solid-state ferroelectric-based synapses for neuromorphic computing52.
Multiferroics
Another field that has hugely benefited from PFM is multiferroics. A combination of PFM and complementary methods, such every bit photoemission electron microscopy (PEEM) and Ten-ray resonant magnetic scattering, enabled the discovery of a local coupling betwixt FE and antiferromagnetic domains in BiFeOiii (BFO) sparse films26 and the emergence of multiferroicity at the interface between ultrathin BaTiO3 films and magnetic layers53. In addition, multi-method approaches involving PFM were used to gain control of the commutation bias in BFO films54 and to switch local ferromagnetism by electric fields55. More than recently, PFM and NV-heart magnetometry have been combined to proceeds an insight into the coupling of the ferroelectric and cycloidal antiferromagnetic domains in BFO56. In multiferroic bulk systems, combinations of PFM and MFM31, magnetoelectric strength microscopy57, transmission electron microscopy (TEM)58, optical microscopy59, also as 2d harmonic generation60, were used to scrutinize the emergence and interplay of structural, electric and magnetic degrees of freedom. In detail, PFM allowed high-resolution imaging of the exotic ferroelectric domain pattern in multiferroic hexagonal manganites61. In multiferroic ceramics, PFM together with complementary scanning TEM measurements was used to demonstrate magnetic-field switching of ferroelectric domains at room temperature (Fig. 3b)62.
Topological states
This new, withal nascent but rapidly growing field addresses vortices, skyrmions, flux closures, chiral, and other topologically protected states in polar materials. In 2004, unusual vortex states were predicted to occur in nanoscale ferroelectrics due to very high depolarization fields63. Just several years afterwards, when advances in processing technologies facilitated the fabrication of such nanometer-scale structures64, vector PFM, as a uniquely suited characterization technique, revealed the predicted emergence of vortex domain states and flux closures (Fig. 3c)65,66. More recently, nanoscale bubble domains, which tin can exist considered as a precursor of electrical skyrmions, have been resolved67.
Functional DWs
Another emerging field extensively relying on PFM focuses on functional DWs in ferroics68, which showroom a range of unusual physical backdrop. The work of Seidel et al.30 stimulated a worldwide interest in DWs by showing that PFM-written DWs in otherwise insulating BFO thin films were electrically conducting. Anisotropic DW conductance was revealed in the improper ferroelectric ErMnO3 (Fig. 3d)69,70. Extended studies on other ferroelectrics showed that unusual DW transport was a rather generic property occurring in a wide range of systems. This includes classical (proper) FEs (PZT71, BaTiOiii 72) besides as improper ferroelectrics RMnOthree (R = Y, In, Dy–Lu)69 (Ca,Sr)iiiTi2O7 73, Cu3B7O13Cl74). The functional properties of DWs and the possibility to inject, erase, or dynamically dope them75 offer unique opportunities for building time to come agile nanoscale devices76. In the start proof-of-principle experiments, PFM helped to demonstrate specific DW-based functionality, using them as spatially moveable nanowires70, digital switches77, and nonvolatile memories78.
Flexoelectricity
Although demonstrated experimentally about fifty years ago, flexoelectricity—a coupling between a polarization and a strain gradient—started to draw much attending only with the appearance of nanotechnology because of larger gradients at the nanoscale79. In ultrathin films, a flexoelectric field due to a strain slope, generated by PFM tip pressure, may even exceed the coercive field leading to purely mechanical switching of polarization (Fig. 3e)lxxx. Nanoscale flexoelectricity has been used for tuning 2d electron gas81, mechanical control of resistive switching37, manipulation of oxygen vacancies82, and even for inducing photovoltaic (PV) effects in centro-symmetric materials83.
Photovoltaics
PVs currently experiences renewed involvement, triggered by the discovery of abnormal PV effects in ferroelectric thin films84. In seminal experiments on BFO, vector PFM was used in combination with macroscopic current–voltage measurements to investigate the affect of the ferroelectric domain geometry on the PV backdrop84. Subsequent in-depth studies85 revealed that the emergent higher up ring-gap voltages originate from the majority PV effect. Furthermore, photocurrents were scrutinized in spatially resolved experiments to clarify the PV/photoconductive properties of the ferroelectric DWs (Fig. 3f)86. In the latest development, PFM allowed demonstration of a tip-enhanced PV effect and light-induced ferroelectric switching87.
Outlook and perspective
We are at an inflection indicate in PFM development and application. On the one hand, PFM has evolved from an imaging technique to a set of advanced methods, which became the prime tools for probing and controlling the static and dynamic properties of nanoscale ferroic structures and devices. On the other manus, a growing range of tested materials revealed PFM limitations: it is now articulate that a careful analysis of the PFM epitome germination mechanism along with comprehensive information on construction, physics, and chemical science of materials obtained by complementary techniques is necessary to distinguish between science and artifacts. Some predictions for future directions and applications of PFM, fabricated several years ago, have not materialized. For case, while PFM can be used every bit a valuable platform for conducting rigorous and replicable testing of new scientific ideas and device concepts, information technology is not likely to go role of an evaluation testbed for integrated memory devices or exist exploited for lesser–up large-scale fabrication of electronic circuits.
Where will it go from hither? Given that the well-nigh important breakthroughs in PFM awarding have been made in combination with other microscopic techniques, it is safe to predict that this trend volition continue. It is envisioned that PFM volition be extended to multiple stimuli (magnetic, optical, thermal) and combinations of those to induce mechanical strain response at a broad range of temporal scales to find emerging phenomena and investigate novel functional properties in a variety of material systems. PFM analysis of the electronic processes in ferroics with respect to the field-induced changes in local construction and chemical composition will exist greatly facilitated by acquisition of the spatially resolved chemical 2D and 3D maps by nano-Raman microscopy and fourth dimension-of-flying secondary ion mass spectroscopy—approaches that accept merely started to gain momentum88. One of the main limitations of PFM volition remain its relatively low time resolution. Short (~ten µm) cantilevers with loftier resonant frequencies (up to several MHz) facilitate video-rate PFM with an imaging speed of nigh 10 frames per 2d. This will allow investigation of domain structure evolution during thermally stimulated phase transitions and relaxation processes on a 100-ms time scale89. However, in application to the fast switching processes, the fourth dimension resolution is unlikely to become below the electric current state-of-the-art nanosecond range of stroboscopic PFM being express by the external circuit time constants. Extension of PFM spectroscopy into the MHz range volition enable drove and multivariate analysis of big amounts of data assuasive a deeper agreement of the underlying mechanisms of the voltage–fourth dimension-dependent switching and energy dissipation processes. 1 of the possible hurdles in big-information analysis could exist the streaming rate of the data.
Realization of DW-based nanotechnology requires awarding of PFM in combination with C-AFM, MFM, scanning impedance microscopy, and nano-Raman spectroscopy complemented by high-resolution electron microscopy studies. This comprehensive approach is desirable to get a ameliorate knowledge of the local electronic states, carrier density contour, mobility and conduction mechanisms, and their relation to the local diminutive structure. Starting time steps in this management have already been made and include local Hall outcome measurementsninety and contact-free loftier-resolution conductance measurements91, equally well as avant-garde nonlinear optical experiments92. Controlled generation and driving of DWs via electric and strain field gradients is a promising arroyo for the realization of DW-based devices and undoubtedly will be explored further.
As a technique, PFM presents a new paradigm in exploring and designing emergent electronic backdrop of advanced oxide, hybrid, and organic nanoferroic materials and nanostructures. State-of-the-fine art PFM methods, in conjunction with first-principle modeling and atomically controlled growth, will play an increasingly important office in investigating the central problems related to the critical behavior and switching dynamics in ultrathin ferroelectric and multiferroic heterostructures. Furthermore, such methods volition let us to empathize the interplay betwixt the ferroelectric and ferromagnetic order parameters, the part of structural defects and interfacial backdrop in the electronic ship at the nanoscale and the scalability of heterostructures to micro-, meso-, and nano-dimensions.
References
-
Kalinin, S. Five. & Gruverman, A. (eds) Scanning Probe Microscopy: Electrical and Electromechanical Phenomena at the Nanoscale (Springer, New York, 2006).
-
Alexe, M. & Gruverman, A. (eds) Ferroelectrics at Nanoscale: Scanning Probe Microscopy Approach (Springer, New York, 2004).
-
Kalinin, S. V., Morozovska, A. Due north., Chen, Fifty. Q. & Rodriguez, B. J. Local polarization dynamics in ferroelectric materials. Rep. Prog. Phys. 73, 056502 (2010). Review article on imaging, manipulation and spectroscopy of ferroelectric switching past PFM with special emphasis on resolution limits .
-
Gruverman, A. & Kholkin, A. Nanoscale ferroelectrics: processing, characterization and hereafter trends. Rep. Prog. Phys. 69, 2443–2474 (2006).
-
Soergel, E. Visualization of ferroelectric domains in bulk single crystals. Appl. Phys. B 81, 729–751 (2005).
-
Kaufman, A. B. Ferroelectric memories for security and identification purposes. IEEE Trans. Electron Devices 16, 562–564 (1969). The concept of polarization reading via the converse piezoelectric effect .
-
Gruverman, A., Auciello, O. & Tokumoto, H. Scanning force microscopy for the written report of domain construction in ferroelectric sparse films. J. Vac. Sci. Technol. B fourteen, 602–605 (1996).
-
Kim, Y. et al. Ionically-mediated electromechanical hysteresis in transition metal oxides. ACS Nano 6, 7026–7033 (2012).
-
Güthner, P. & Dransfeld, G. Local poling of ferroelectric polymers past scanning force microscopy. Appl. Phys. Lett. 61, 1137–1139 (1992).
-
Güthner, P., Glatz-Reichenbach, J. & Dransfeld, K. Investigation of local piezoelectric properties of sparse copolymer films. J. Appl. Phys. 69, 7895–7897 (1991).
-
Birk, H., Glatz-Reichenbach, J., Jie, L., Schreck, Eastward. & Dransfeld, K. The local piezoelectric activity of thin polymer films observed by scanning tunneling microscopy. J. Vac. Sci. Technol. B 9, 1162–1165 (1991).
-
Cross, 50. E. & Newnham, R. E. High-Technology Ceramics (American Ceramic Society, Ohio, 1987).
-
Gruverman, A., Auciello, O. & Tokumoto, H. Nanoscale investigation of fatigue effects in Atomic number 82(Zr,Ti)O3 films. Appl. Phys. Lett. 69, 3191–3193 (1996).
-
Alexe, M., Harnagea, C., Hesse, D. & Gosele, U. Patterning and switching of nanosize ferroelectric retention cells. Appl. Phys. Lett. 75, 1793–1795 (1999).
-
Tybell, T., Ahn, C. H. & Triscone, J.-M. Ferroelectricity in thin perovskite films. Appl. Phys. Lett. 75, 856–858 (1999).
-
Ganpule, C. South. et al. Scaling of ferroelectric and piezoelectric backdrop in Pt/SrBi2Ta2O9/Pt thin films. Appl. Phys. Lett. 75, 3874–3876 (1999).
-
Luo, Y. et al. Nanoshell tubes of ferroelectric lead zirconate titanate and barium titanate. Appl. Phys. Lett. 83, 440–442 (2003).
-
Eng, L. Chiliad. Nanoscale domain engineering and label of ferroelectric domains. Nanotechnology 10, 405–411 (1999).
-
Jesse, S., Baddorf, A. P. & Kalinin, S. V. Switching spectroscopy piezoresponse force microscopy of ferroelectric materials. Appl. Phys. Lett. 88, 062908 (2006).
-
Hong, South. et al. Loftier resolution written report of domain nucleation and growth during polarization switching in Pb(Zr,Ti)O3 ferroelectric sparse motion-picture show capacitors. J. Appl. Phys. 86, 607–613 (1999).
-
Gruverman, A., Wu, D. & Scott, J. F. Piezoresponse force microscopy studies of switching beliefs of ferroelectric capacitors on a 100-ns time scale. Phys. Rev. Lett. 100, 097601 (2008). First direct observation of domain nucleation and domain wall dynamics at the sub-100-ns fourth dimension scale in micrometer-size device-class PZT capacitors .
-
Kalinin, Due south. V. et al. Vector piezoresponse forcefulness microscopy. Microsc. Microanal. 12, 206–220 (2006).
-
Eng, L. K., Güntherodt, H.-J., Schneider, G. A., Köpke, U. & Muñoz Saldaña, J. Nanoscale reconstruction of surface crystallography from 3-dimensional polarization distribution in ferroelectric barium–titanate ceramics. Appl. Phys. Lett. 74, 233–235 (1999).
-
Kalinin, South. Five. et al. Imaging machinery of piezoresponse force microscopy in capacitor structures. Appl. Phys. Lett. 92, 152906 (2008).
-
Harnagea, C., Pignolet, A., Alexe, M. & Hesse, D. Piezoresponse scanning force microscopy: what quantitative information tin we really get out of piezoresponse measurements on ferroelectric thin films. Integr. Ferroelectr. 38, 23–29 (2001). Introduction of the method to relate the PFM amplitude to the ferroelectric polarization value while taking into account the anisotropic nature of the piezoelectric tensor and polarization orientation .
-
Zhao, T. et al. Electric command of antiferromagnetic domains in multiferroic BiFeO3 films at room temperature. Nat. Mater. v, 823–829 (2006). First demonstration of electrical control of antiferromagnetic domain structure in a single-phase multiferroic textile .
-
Yoshida, C., Yoshida, A. & Tamura, H. Nanoscale conduction modulation in Au/Pb(Zr, Ti)O3/SrRuOiii heterostructure. Appl. Phys. Lett. 75, 1449–1451 (1999).
-
Garcia, Five. et al. Giant tunnel electroresistance for non-subversive readout of ferroelectric states. Nature 460, 81–84 (2009). First sit-in of polarization-controlled tunnel electroresistance issue using a combination of PFM and C-AFM .
-
Gruverman, A. et al. Tunneling electroresistance effect in ferroelectric tunnel junctions at the nanoscale. Nano Lett. 9, 3539–3543 (2009).
-
Seidel, J. et al. Conduction at domain walls in oxide multiferroics. Nat. Mater. 8, 229–234 (2009). Start sit-in of domain wall conductivity using a combination of PFM and C-AFM .
-
Geng, Y., Lee, N., Choi, Y. J., Cheong, S. W. & Wu, Due west. Commonage magnetism at multiferroic vortex domain walls. Nano Lett. 12, 6055–6059 (2012).
-
Denning, D., Guyonnet, J. & Rodriguez, B. J. Applications of piezoresponse strength microscopy in materials research: from inorganic ferroelectrics to biopiezoelectrics and across. Int. Mater. Rev. 61, 46–70 (2016).
-
Rodriguez, B. J., Callahan, C., Kalinin, South. V. & Proksch, R. Dual-frequency resonance-tracking diminutive force microscopy. Nanotechnology 18, 475504 (2007).
-
Harnagea, C., Alexe, Thou., Hesse, D. & Pignolet, A. Contact resonances in voltage-modulated force microscopy. Appl. Phys. Lett. 83, 338–340 (2003).
-
Borowiak, A. Southward. et al. Electromechanical response of amorphous LaAlO3 sparse picture show probed by scanning probe microscopies. Appl. Phys. Lett. 105, 012906 (2014).
-
Bark, C. W. et al. Switchable induced polarization in LaAlOthree/SrTiO3 heterostructures. Nano Lett. 12, 1765–1771 (2012).
-
Kim, Y. et al. Mechanical control of electroresistive switching. Nano Lett. xiii, 4068–4074 (2013).
-
Seol, D., Kim, B. & Kim, Y. Not-piezoelectric furnishings in piezoresponse force microscopy. Curr. Appl. Phys. 17, 661–674 (2017).
-
Miao, H., Tan, C., Zhou, Ten., Wei, Ten. & Li, F. More ferroelectrics discovered past switching spectroscopy piezoresponse force microscopy? EPL 108, 27010 (2014).
-
Hermes, I. M. et al. Ferroelastic fingerprints in methylammonium atomic number 82 iodide perovskite. J. Phys. Chem. C 120, 5724–5731 (2016).
-
Kutes, Y. et al. Directly observation of ferroelectric domains in solution-processed CHthreeNH3PbIiii perovskite sparse films. J. Phys. Chem. Lett. 5, 3335–3339 (2014).
-
Balke, Northward. et al. Differentiating ferroelectric and nonferroelectric electromechanical furnishings with scanning probe microscopy. ACS Nano 9, 6484–6492 (2015). Consideration of various mechanisms of the PFM paradigm germination .
-
Kalinin, S. Five. & Bonnell, D. A. Imaging machinery of piezoresponse force microscopy of ferroelectric surfaces. Phys. Rev. B. 65, 125408 (2002).
-
Soergel, E. Piezoresponse strength microscopy (PFM). J. Phys. D Appl. Phys. 44, 464003 (2011).
-
Balke, N. et al. Quantification of surface displacements and electromechanical phenomena via dynamic atomic force microscopy. Nanotechnology 27, 425707 (2016).
-
Morozovska, A. Northward. et al. Piezoresponse force spectroscopy of ferroelectric-semiconductor materials. J. Appl. Phys. 102, 114108 (2007).
-
Dahan, D. et al. Ferroelectric domain inversion: the role of humidity. Appl. Phys. Lett. 89, 152902 (2006).
-
Chanthbouala, A. et al. Solid-land memories based on ferroelectric tunnel junctions. Nat. Nano 7, 101–104 (2012).
-
Pantel, D., Goetze, S., Hesse, D. & Alexe, Chiliad. Reversible electrical switching of spin polarization in multiferroic tunnel junctions. Nat. Mater. 11, 289–293 (2012).
-
Chanthbouala, A. et al. A ferroelectric memristor. Nat. Mater. 11, 860–864 (2012).
-
Kim, D. J. et al. Ferroelectric tunnel memristor. Nano Lett. 12, 5697–5702 (2012).
-
Boyn, S. et al. Learning through ferroelectric domain dynamics in solid-state synapses. Nat. Commun. viii, 14736 (2017).
-
Valencia, S. et al. Interface-induced room-temperature multiferroicity in BaTiO3. Nat. Mater. ten, 753–758 (2011).
-
Martin, L. Westward. et al. Nanoscale control of commutation bias with BiFeOthree thin films. Nano Lett. eight, 2050–2055 (2008).
-
Chu, Y.-H. et al. Electric-field control of local ferromagnetism using a magnetoelectric multiferroic. Nat. Mater. 7, 478–482 (2008).
-
Gross, I. et al. Real-space imaging of not-collinear antiferromagnetic order with a single-spin magnetometer. Nature 549, 252–256 (2017).
-
Geng, Y. et al. Straight visualization of magnetoelectric domains. Nat. Mater. xiii, 163–167 (2014).
-
Choi, T. et al. Insulating interlocked ferroelectric and structural antiphase domain walls in multiferroic YMnOthree. Nat. Mater. 9, 253–258 (2010).
-
Chae, South. C. et al. Direct observation of the proliferation of ferroelectric loop domains and vortex-antivortex pairs. Phys. Rev. Lett. 108, 167603 (2012).
-
Lilienblum, Thousand. et al. Ferroelectricity in the multiferroic hexagonal manganites. Nat. Phys. 11, 1070–1073 (2015).
-
Jungk, T., Hoffmann, Á., Fiebig, 1000. & Soergel, E. Electrostatic topology of ferroelectric domains in YMnOiii. Appl. Phys. Lett. 97, 012904 (2010).
-
Evans, D. Thou. et al. Magnetic switching of ferroelectric domains at room temperature in multiferroic PZTFT. Nat. Commun. iv, 1534 (2013).
-
Naumov, I. I., Bellaiche, L. & Fu, H. Unusual stage transitions in ferroelectric nanodisks and nanorods. Nature 432, 737–740 (2004).
-
Lee, West. et al. Individually addressable epitaxial ferroelectric nanocapacitor arrays with most Tb inch−2 density. Nat. Nanotechnol. three, 402–407 (2008).
-
Ivry, Y., Chu, D. P., Scott, J. F. & Durkan, C. Flux closure vortex-similar domain structures in ferroelectric thin films. Phys. Rev. Lett. 104, 207602 (2010).
-
McQuaid, R. M. P., McGilly, L. J., Sharma, P., Gruverman, A. & Gregg, J. Thou. Mesoscale flux-closure domain germination in unmarried-crystal BaTiO3. Nat. Commun. 2, 404 (2011).
-
Zhang, Q. et al. Nanoscale bubble domains and topological transitions in ultrathin ferroelectric films. Adv. Mater. 29, 1702375 (2017).
-
Catalan, Yard., Seidel, J., Ramesh, R. & Scott, J. F. Domain wall nanoelectronics. Rev. Mod. Phys. 84, 119–156 (2012). Key review commodity on domain walls highlighting the office of PFM in delineating their properties .
-
Meier, D. et al. Anisotropic conductance at improper ferroelectric domain walls. Nat. Mater. 11, 284–288 (2012).
-
Schaab, J. et al. Optimization of electronic domain-wall properties by aliovalent cation substitution. Adv. Electron. Mater. 2, 1500195 (2016).
-
Guyonnet, J., Gaponenko, I., Gariglio, S. & Paruch, P. Conduction at domain walls in insulating Pb(Zr0.iiTi0.8)O3 thin films. Adv. Mater. 23, 5377–5382 (2011).
-
Sluka, T., Tagantsev, A. G., Bednyakov, P. & Setter, Northward. Free-electron gas at charged domain walls in insulating BaTiOiii. Nat. Commun. 4, 1808 (2013).
-
Oh, Y. S., Luo, X., Huang, F.-T., Wang, Y. & Cheong, Due south.-Due west. Experimental sit-in of hybrid improper ferroelectricity and the presence of arable charged walls in (Ca,Sr)threeTitwoOvii crystals. Nat. Mater. fourteen, 407–413 (2015).
-
McQuaid, R. Thou. P., Campbell, M. P., Whatmore, R. W., Kumar, A. & Gregg, J. M. Injection and controlled motility of conducting domain walls in improper ferroelectric Cu-Cl boracite. Nat. Commun. 8, 15105 (2017).
-
Whyte, J. R. et al. Ferroelectric domain wall injection. Adv. Mater. 26, 293–298 (2014).
-
Whyte, J. R. & Gregg, J. M. A diode for ferroelectric domain-wall motion. Nat. Commun. six, 7361 (2015).
-
Mundy, J. A. et al. Functional electronic inversion layers at ferroelectric domain walls. Nat. Mater. sixteen, 622–627 (2017).
-
Sharma, P. et al. Nonvolatile ferroelectric domain wall memory. Sci. Adv. three, e1700512 (2017).
-
Zubko, P., Catalan, G. & Tagantsev, A. K. Flexoelectric effect in solids. Annu. Rev. Mater. Res. 43, 387–421 (2013).
-
Lu, H. et al. Mechanical writing of ferroelectric polarization. Science 336, 59–61 (2012). Pioneering observation of purely mechanical 180° switching of polarization via flexoelectric effect .
-
Sharma, P. et al. Electromechanics of ferroelectric-like behavior of LaAlOthree sparse films. Adv. Funct. Mater. 25, 6538–6544 (2015).
-
Das, S. et al. Controlled manipulation of oxygen vacancies using nanoscale flexoelectricity. Nat. Commun. 8, 615 (2017).
-
Yang, M. 1000., Kim, D. J. & Alexe, M. Flexo-photovoltaic effect. Science 360, 904–907 (2018). The majority photovoltaic effect can be realized in any semiconductor, including silicon, via the flexoelectric effect .
-
Yang, S. Y. et al. Above-bandgap voltages from ferroelectric photovoltaic devices. Nat. Nanotechnol. five, 143–147 (2010).
-
Bhatnagar, A., Roy Chaudhuri, A., Heon Kim, Y., Hesse, D. & Alexe, K. Function of domain walls in the abnormal photovoltaic effect in BiFeO3. Nat. Commun. four, 2835 (2013).
-
Yang, M.-M., Bhatnagar, A., Luo, Z.-D. & Alexe, Chiliad. Enhancement of local photovoltaic current at ferroelectric domain walls in BiFeO3. Sci. Rep. 7, 43070 (2017).
-
Yang, M. M. & Alexe, M. Light-induced reversible control of ferroelectric polarization in BiFeO3. Adv. Mater. xxx, 1704908 (2018). Demonstration of calorie-free-induced ferroelectric switching .
-
Ievlev, A. V. et al. Chemical state evolution in ferroelectric films during tip-induced polarization and electroresistive switching. ACS Appl. Mater. Interfaces eight, 29588–29593 (2016).
-
Nath, R., Chu, Y.-H., Polomoff, N. A., Ramesh, R. & Huey, B. D. High speed piezoresponse force microscopy: <1 frame per second nanoscale imaging. Appl. Phys. Lett. 93, 072905 (2008).
-
Campbell, Thou. P. et al. Hall outcome in charged conducting ferroelectric domain walls. Nat. Commun. 7, 13764 (2016).
-
Schaab, J. et al. Contact-free mapping of electronic ship phenomena of polar domains in SrMnOiii films. Phys. Rev. Appl. 5, 054009 (2016).
-
De Luca, G. et al. Domain wall architecture in tetragonal ferroelectric thin films. Adv. Mater. 29, 1605145 (2017).
-
Kim, D. J. et al. Observation of inhomogeneous domain nucleation in epitaxial Pb(Zr,Ti)O3 capacitors. Appl. Phys. Lett. 91, 132903 (2007).
-
Gruverman, A., Cross, J. S. & Oates, W. S. Peculiar effect of mechanical stress on polarization stability in micrometer-calibration ferroelectric capacitors. Appl. Phys. Lett. 93, 242902 (2008).
-
Jesse, Due south. et al. Direct imaging of the spatial and free energy distribution of nucleation centers in ferroelectric materials. Nat. Mater. vii, 209–215 (2008).
-
Park, K. et al. Three-dimensional ferroelectric domain imaging of epitaxial BiFeO3 thin films using angle-resolved piezoresponse force microscopy. Appl. Phys. Lett. 97, 112907 (2010).
-
Labuda, A. & Proksch, R. Quantitative measurements of electromechanical response with a combined optical beam and interferometric atomic strength microscope. Appl. Phys. Lett. 106, 253103 (2015).
-
Jesse, Southward., Kalinin, Due south. V., Proksch, R., Baddorf, A. P. & Rodriguez, B. J. The band excitation method in scanning probe microscopy for rapid mapping of energy dissipation on the nanoscale. Nanotechnology 18, 435503 (2007).
-
Peter, F. et al. Contributions to in-plane piezoresponse on axially symmetrical samples. Rev. Sci. Instrum. 76, 106108 (2005).
Acknowledgements
A.G. acknowledges support past the National Scientific discipline Foundation through Materials Research Science and Technology Center nether Grant No. DMR-0820521. M.A. acknowledges support of Royal Society through the Wolfson Inquiry Merit and Theo Irish potato Bluish-heaven Awards and EPSRC (UK) through Grant Nos. EP/P031544/i and EP/P025803/one. D.Chiliad. thank you NTNU for support through the Onsager Fellowship Programme and the Outstanding Academic Fellows Programme. The authors thank Steffen Brosseit (Brossboss Entertainment) for animating Fig. 1a, b.
Author information
Affiliations
Contributions
All authors contributed to all aspects of this piece of work.
Corresponding author
Ethics declarations
Competing interests
The authors declare no competing interests.
Boosted data
Journal peer review information: Nature Communications thanks Colm Durkan and the other anonymous reviewers for their contribution to the peer review of this work.
Publisher'south note: Springer Nature remains neutral with regard to jurisdictional claims in published maps and institutional affiliations.
Rights and permissions
Open Admission This article is licensed nether a Artistic Commons Attribution 4.0 International License, which permits use, sharing, adaptation, distribution and reproduction in whatever medium or format, every bit long as y'all give advisable credit to the original author(s) and the source, provide a link to the Creative Commons license, and indicate if changes were made. The images or other third party textile in this article are included in the article's Creative Eatables license, unless indicated otherwise in a credit line to the cloth. If material is not included in the commodity'due south Creative Commons license and your intended use is non permitted by statutory regulation or exceeds the permitted use, you volition demand to obtain permission directly from the copyright holder. To view a copy of this license, visit http://creativecommons.org/licenses/by/4.0/.
Reprints and Permissions
Almost this commodity
Cite this article
Gruverman, A., Alexe, M. & Meier, D. Piezoresponse force microscopy and nanoferroic phenomena. Nat Commun 10, 1661 (2019). https://doi.org/10.1038/s41467-019-09650-eight
-
Received:
-
Accustomed:
-
Published:
-
DOI : https://doi.org/10.1038/s41467-019-09650-eight
Further reading
-
Direct ascertainment of ferroelectricity in two-dimensional MoS2
npj 2d Materials and Applications (2022)
-
Scanning probe microscopy
Nature Reviews Methods Primers (2021)
-
Piezoelectricity in hafnia
Nature Communications (2021)
-
Interfacial piezoelectric polarization locking in printable Ti3C2Tx MXene-fluoropolymer composites
Nature Communications (2021)
-
Ion adsorption-induced reversible polarization switching of a van der Waals layered ferroelectric
Nature Communications (2021)
Comments
By submitting a annotate yous agree to bide past our Terms and Community Guidelines. If you find something abusive or that does not comply with our terms or guidelines please flag it as inappropriate.
Source: https://www.nature.com/articles/s41467-019-09650-8
0 Response to "Nanoferroics State of Art Gradient Driven Couplings and Advanced Applications"
Post a Comment